INTRODUCTION
If you have ever observed the night sky, you surely know that it swarms with countless stars of various sizes. It is remarkable when we realise that the light of these stars has travelled many years through space-time before it reached our minuscule blue planet and hit the retina of our eyes, where we are able to detect it and interpret its originator as a peculiar tiny dot in the sky. But what is more, the light from the stars we can observe today is often even several centuries old – this is how long it takes for light to travel the enormous distances that separate us from these stars. 1 Observing the night sky is therefore in its essence like traveling into the past. Who knows, some of the stars in today’s night sky may not even exist anymore.
But even more remarkable is the immense number of stars. If you happen to be very lucky and observe the night sky far away from the cities’ light pollution, you may behold up to 2000 diminutive dots. That may seem like an impressive number, but it is only one fifty-millionth of all the stars hiding in the heart of our galaxy. The Milky Way contains an estimated number of staggering 100 billion stars, our Sun of course being one of them. If we add all the other stars from billions and billions various galaxies of the universe, we get a truly incredible number. The entire observable universe might hold up to 100 billion billion billion stars! 2
![]() |
![]() |
![]() |
![]() |
And each star is a little unique. Some finish their lives in a massive explosion, others leave this world in a considerably more peaceful manner. Stars are mesmerizing and omnipresent inhabitants of the cosmos, without which life could not possibly arise. Therefore it is appropriate to understand them at least a little bit.
The life of stars begins in huge clouds (nebulae) made predominantly from the lightest elements. These cosmic clouds perpetually come into contract due to gravity, which gradually raises their temperature. Once the temperature of the nebula reaches a sufficient value, the electrons inside of it decide that they no longer wish to form atoms and a peculiar state of matter called plasma is created. 3 At this moment, the interstellar cloud consists of negatively charged electrons separated from positively charged atomic nuclei.
These hydrogen nuclei then move fiercely throughout the nebula and often come across different nuclei. But once two nuclei get too close to one another, electromagnetic interaction starts showing and swiftly splits them apart again. However, we should not forget that the temperature of the cloud still rises thanks to gravity. Eventually, it raises to such an extent that the nuclei manage to trick the electromagnetic force. The velocity of individual nuclei grows with the temperature of the cloud, so in the end they are able to overcome the immense repulsion of electromagnetism by getting so close that the enormous power of the strong interaction shows itself, and the nuclei are united into a single helium nucleus. At this moment, nuclear fusion has just began in the nebula, which can only mean one thing – a star has been born.
Our newly created star then continues with nuclear fusion, which becomes the source of tremendous energy. Due to this energy, the star is able to stop its own gravitational collapse – up to this moment, the original cloud (star) kept shrinking. Thanks to the energy from fusion, the star is able to create photons – the particles of light, which give stars their distinctive glow. Each star sends off billions of photons into the surrounding cosmos every second. These photons then travel freely through space-time until they reach an impediment that would absorb them and steal their energy.
Sometimes we do not even realize how dependent we actually are on our parental star’s photons. If the Sun suddenly stopped suppling us with its precious light, the Earth would change dramatically in no time. Eight minutes and twenty seconds after the Sun’s extinction, the Earth would submerge into an eternal darkness.
The temperature would fall beyond the freezing point in just a week, which would cause the freezing of all world’s oceans – water in its liquid form would exist just near the ocean floor, due to the heat from the Earth’s heart. Plants would immediately stop producing atmospheric oxygen by photosynthesis, and they would die shortly thereafter. This would cause starvation and early death of all herbivores. Carnivores and omnivores would follow in just a moment – including humans, understandably.
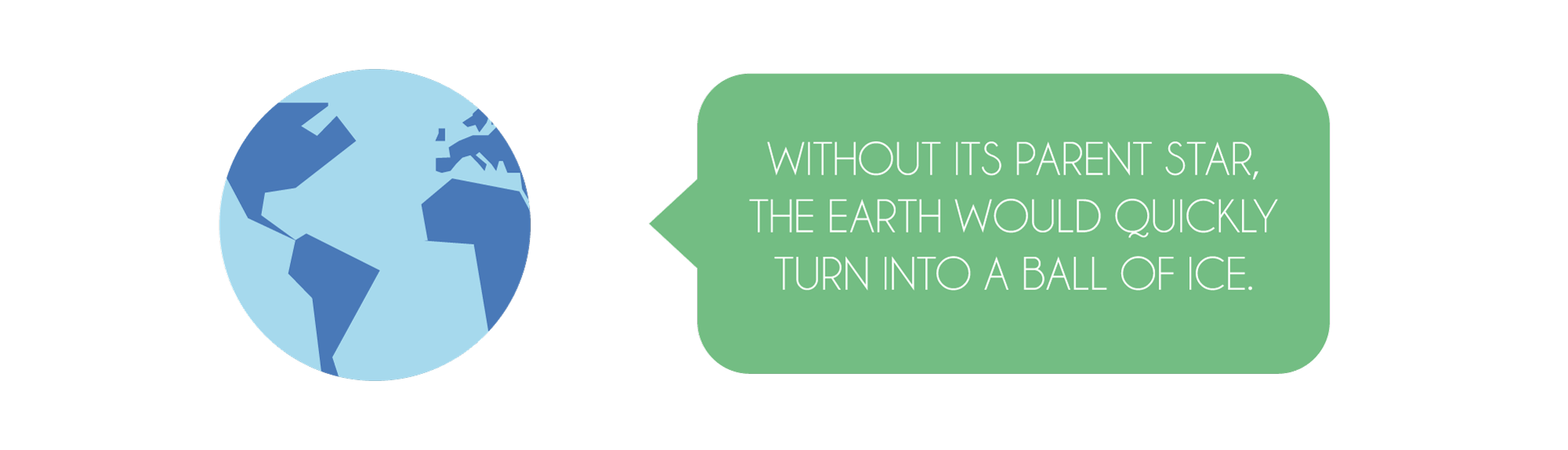
The differences in the Earth’s atmosphere would even out before long, any kind of wind would therefore cease to exist. The same goes for all the rivers of the world, since it would never rain again. All of these huge changes would significantly limit our last chance of survival – the production of electric energy. It is reasonable to assume that only a handful of lucky individuals would be able to survive, though not for long. All the remaining life on Earth would be concentrated at the bottom of the oceans. The Earth would become a dim and eternally frozen wasteland.
However, we do not have to worry about anything like that – for now. The Sun is about to stay here with us for at least a few billion years. But not all stars are this lucky. Some only live a fraction of our closest star’s life.
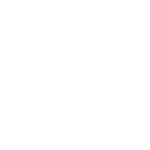
![]() |
NEXT |
STELLAR CLASIFICATION
The estimation of a star’s life expectancy has turned out to be quite simple. It only depends on one property – mass. The mass of a star determines all significant properties of the star – the aforementioned life expectancy, but also colour, temperature and obviously size. It also specifies its destiny. Let us focus on this chart now, which is known under the name of HR Diagram. This diagram is crucial for a deeper understanding of stars. With a bit of courage, it might even be said that the HR Diagram is one of the most eminent findings of astronomy:
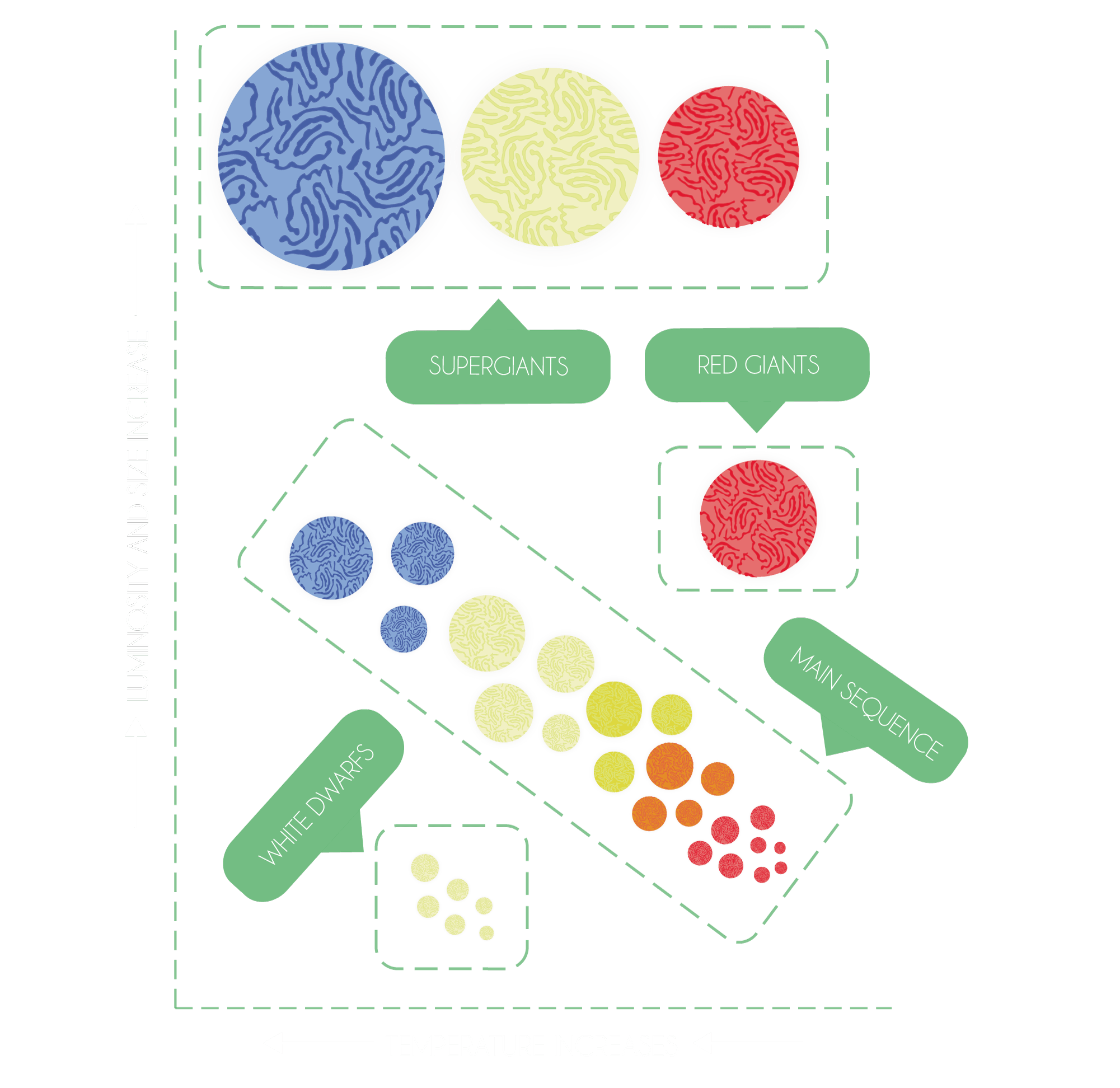
The diagram in front of your eyes classifies all stars in the universe – each star in the observable cosmos could be categorized into one of the categories above. First, let us focus on the most important part of the diagram, the main sequence, which stretches diagonally from the top left-hand corner to the bottom right-hand corner. At the start of its life, every star belongs among the stars of the main sequence. All the stars outside this sequence are either dying (giants and supergiants) or have been long dead and just keep harvesting the energy they have produced during their lifetime (white dwarfs).
Let us now describe the fascinating life story of individual star types. And let us begin with the very smallest ones, which a located at the bottom right-hand corner of our diagram. These stars have earned an apt nickname – red dwarfs.
The life of a red dwarf starts the same as the life of all the other stars – inside of a nebula. For some reason, however, red dwarfs have not been able to accumulate as much matter as their larger relatives – perhaps their nebula was too small or a different star forming in the same nebula has deprived them of some mass. Whatever the case, the mass of a red dwarf ranges from 0.1 to 0.5 solar masses. 4 Their red colour comes from the fact that they have quite a low temperature, so they only emit red light, which has the lowest energy. 5 What is truly remarkable is that more than three quarters of all stars in our galaxy happen to be red dwarfs.
However, if you wish to set your eyes on one of them, I have bad news for you. From the Earth, we cannot observe even a single red dwarf, since they have very small luminosity – sometimes even one ten-thousandth of the Sun’s luminosity. They compensate for this deficit by being able to glow for trillions of years. Red dwarfs are true masters of longevity – since the birth of the cosmos, not even one of them has used up its fuel in the form of hydrogen. Therefore, we cannot know how the life of a red dwarf ends – not even one of them has died to this day. But very likely they will end up in the bottom left-hand corner of our diagram as white dwarfs, where they will stay for another billions of years.
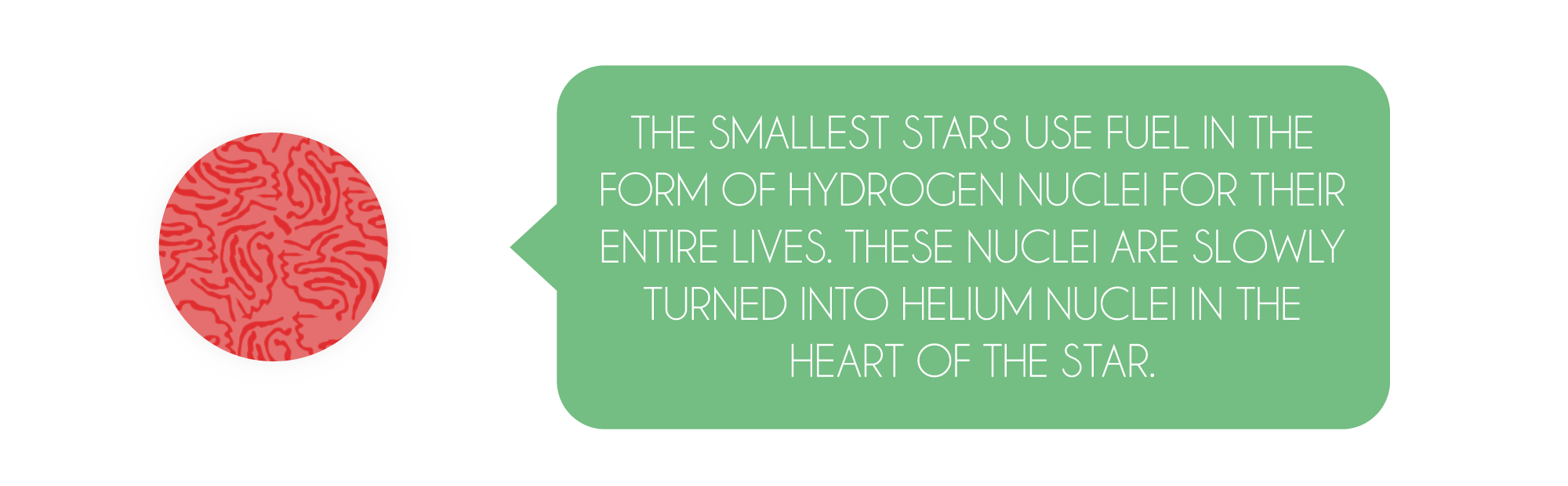
Now, let us move to the next category of stars. Stars of similar masses as our Sun (located in the middle of the main sequence) leave the world in a somewhat more spectacular way. At the beginning of their lives, Sun-like stars behave just like red dwarfs – they serenely link hydrogen nuclei and radiate heat. But they have much greater temperature, which gives them their yellow or white colour. 6
However, as a sacrifice for their greater luminosity, they die much faster. Once a Sun-like star depletes the hydrogen supplies in its centre, which usually lasts several billion years, the core begins to collapse rapidly under its huge gravitational pressure. The gravitational crash causes a massive rise in the core’s temperature – sometimes even a hundred million degrees Celsius. However, this temperature is sufficient for the star to start connecting helium nuclei (the second lightest element) in their cores and therefore avert its extinction temporarily.
But this trick has a little side effect. As the star starts fusing helium, its blistering core starts warming the surrounding parts, which causes a massive rise in the star’s volume and eventually its transformation into a red giant (the top right-hand corner of the diagram). It is appropriate to know one thing about red giants – the term giant is definitely not exaggerated.
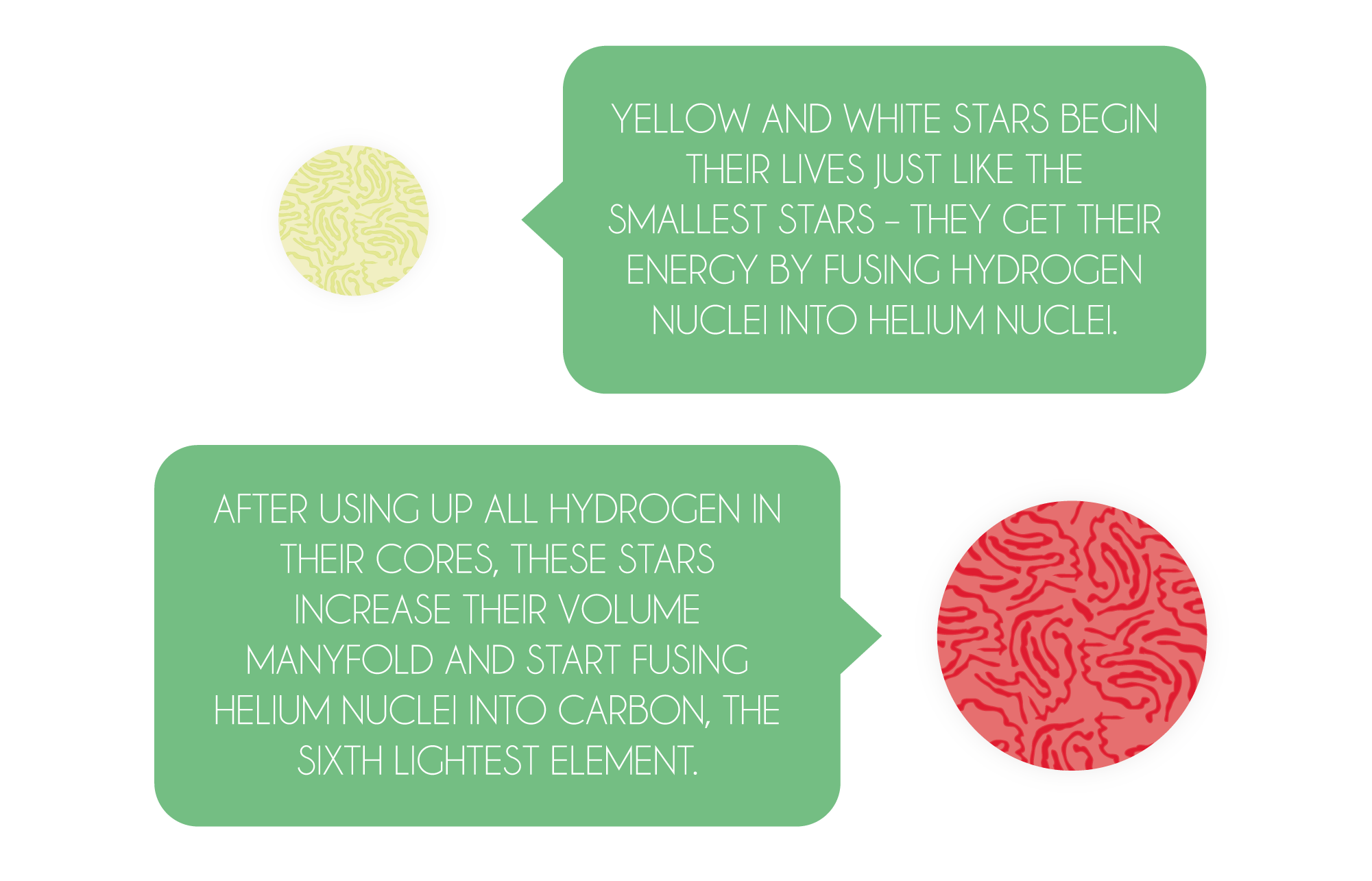
It is estimated that once the Sun gets to the red giant phase, it will increase its diameter about 250 times. Imagine a football that just so happens to inflate to the size of a huge sphere 60 meters in diameter – a similar thing will happen to our Sun. It goes without saying that when it happens, it will not be a good idea linger anywhere in the vicinity of the Earth, unless you wish to be burned alive (the heat will become so intense that even mountains will begin to melt) or even pulled inside the Sun’s interior – scientists are still unable to agree as to whether the Earth will be at least partially spared from the Sun’s rampage, or whether it will be engulfed along with the first two planets of our solar system – Mercury and Venus.
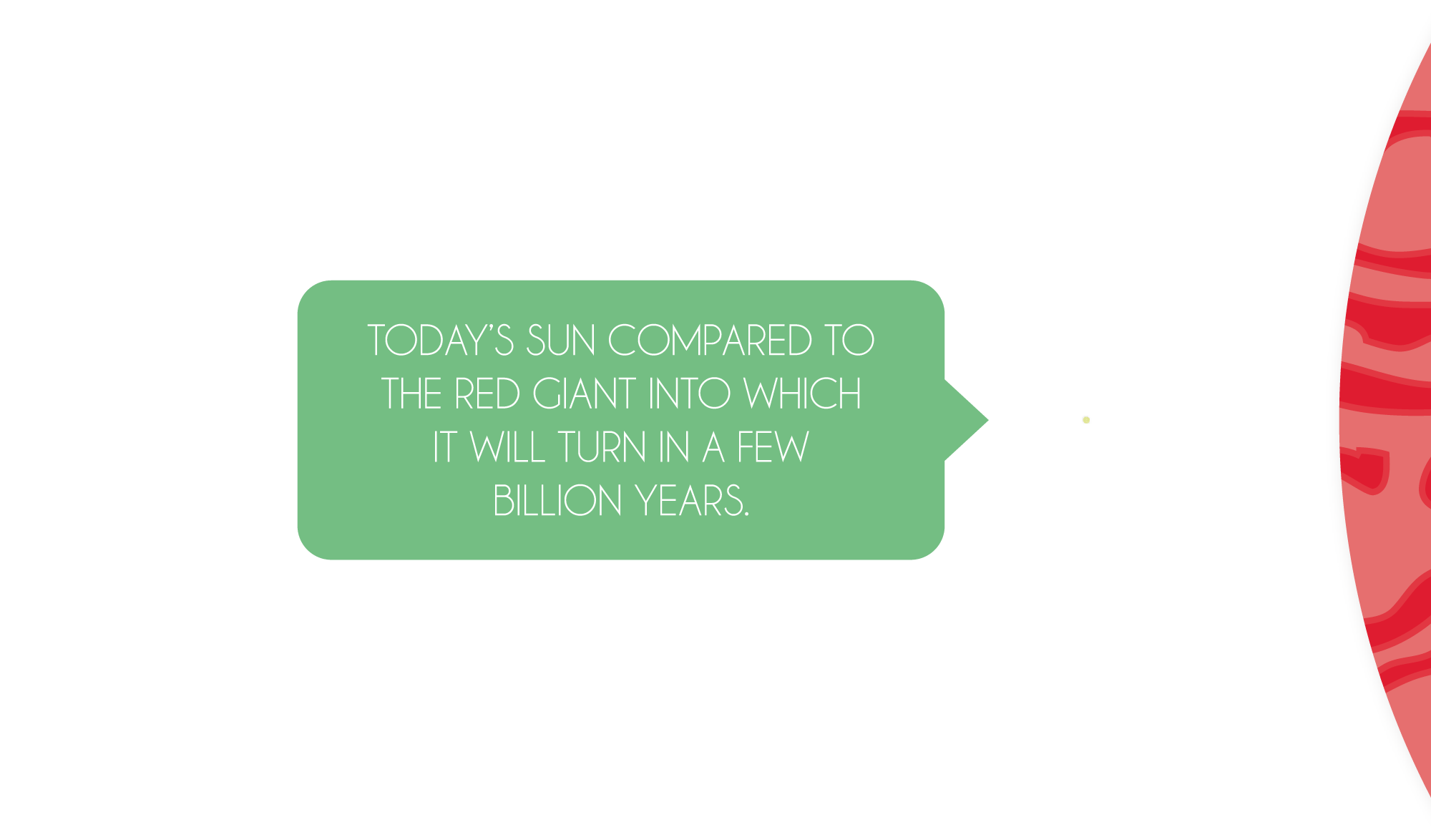
Whatever the case, if the human civilisation is still around at this point it time, it will roam a different part of the universe – perhaps humans will find a new home somewhere around Pluto, which will at that time provide a convenient temperature for the existence of water in its liquid form. But maybe they will depart to a completely different corner of the Milky Way galaxy. The only consolation provides the fact that it will take several billion years before our Sun swells so much. But let us go back to red giants.
Once a red giant exhausts even all of its helium, it spontaneously ejects billions of tons of material into the surrounding cosmos, and the sole thing left from the entire star will be its core in the form of a white dwarf. An average white dwarf is not much bigger than our own planet. Stars that used to be grandiose red giants therefore end their lives in the same way as red dwarfs – as relatively small space objects waiting to cool down. The whole life of such a star, from its birth to its majestic transition into a red dwarf, lasts a few billion years. If we compare that to those trillions of years red dwarfs can be proud of, we might even say that stars originating from the very centre of the main sequence do not seem to be particularly teemed with longevity.
Now, let us focus our attention on the most remarkable of stars – massive blue stars from the top left-hand corner of the main sequence. The lower limit of their mass is about ten solar masses, but some can reach up to a hundred solar masses. These stars are incredibly luminescent – sometimes even a million times more than the Sun. For this reason, they quickly 7 burn all of their fuel – a star fifty times more massive than the Sun exhausts all of its hydrogen in “just” one hundred million years. After that, blue stars use the same trick as their smaller companions and start fusing helium atoms as giants.
But their pilgrimage is far from finishing here. Once they burn all the helium in their cores, they move to the next stage of their lives, a stage that is even more impressive than the previous one – they become supergiants. It goes without saying that supergiants are enormous. Their diameter is often even a thousand times greater than that of the Sun (and a hundred thousand times greater than the diameter of our tiny planet).
Due to the huge temperature in their cores, supergiants present determined machineries with only one purpose – to assemble heavier elements. While lighter and cooler stars are only able to fuse helium nuclei, which makes them capable of creating carbon nuclei at most, supergiants are definitely not frightened to create even much heavier nuclei. Their endeavour does not stop until it reaches iron – the 26th lightest element. Iron presents the final milestone for fusion. The arrangement of particles in iron’s nucleus is so energetically efficient that whenever iron fuses with a different nucleus, energy is consumed, not created.
At iron, supergiants therefore reach the fatal breaking point – the only efficient tool they have had to counteract gravity betrays them, which means they can no longer resist their gravitational collapse. The atmosphere of the star starts to drift towards its heart under an incredible velocity. After a few minutes, it reaches the star’s nucleus. Once there, the whole atmosphere is bounced back with a tremendous power. The result is an immense explosion in the form of a supernova.
Imagine the overwhelming energy of the nuclear bomb that wiped Hiroshima off the face of the Earth back in 1945. Now multiply that energy million million million million times. Why? Such is the energy released during a supernova explosion. This stellar explosion is so enormous that it cannot possibly be expressed by any superlative. A supernova can outshine the collective brightness of all other stars in its entire home galaxy.
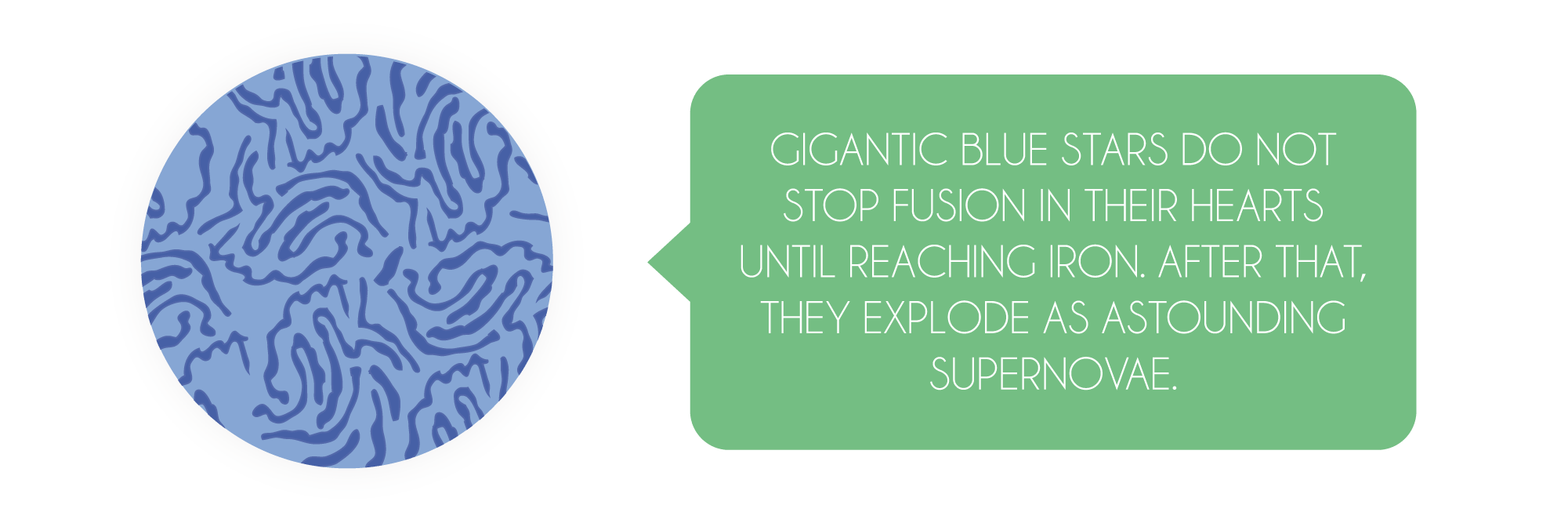
During the explosion, the star has so much redundant energy that it even starts producing elements that are heavier than iron. An average supernova explosion takes only about a minute. In such a brief period of time, an exploding star is able to assemble all of 92 naturally occurring elements of the universe. Heavy elements, such as zinc, iodine, or uranium would never see the light of day were it not for the staggering supernova explosions, since nothing else in the universe simply has the amount of energy necessary for their construction. It is mesmerizing when we realise that all heavier elements around you have once been ejected by one of the ancient supernovae of the early universe.
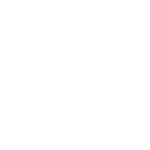
![]() |
![]() |
![]() |
PREVIOUS |
SHARE |
NEXT |
THE EXTREMES OF THE COSMOS
Before we can focus on what happens to the core of a star during a supernova explosion, we first need to become closely acquainted with atoms. As I have already mentioned in previous chapters, each atom consists of a nucleus and a surrounding shell. Protons and neutrons are located in the core, while electrons move randomly around the shell. An important fact is that nearly all energy of an atom is concentrated in its core – protons and neutrons are almost 2000 times more massive than electrons.
Another important fact is that the core is incredibly small compared to the size of the entire atom. For instance, the radius of a hydrogen nucleus is 145 000 times smaller than the radius of the whole hydrogen atom. This means that atoms are mostly empty space. There is an extremely dense and incredibly small nucleus in the centre, surrounded by a nearly empty shell, where an electron occasionally appears.
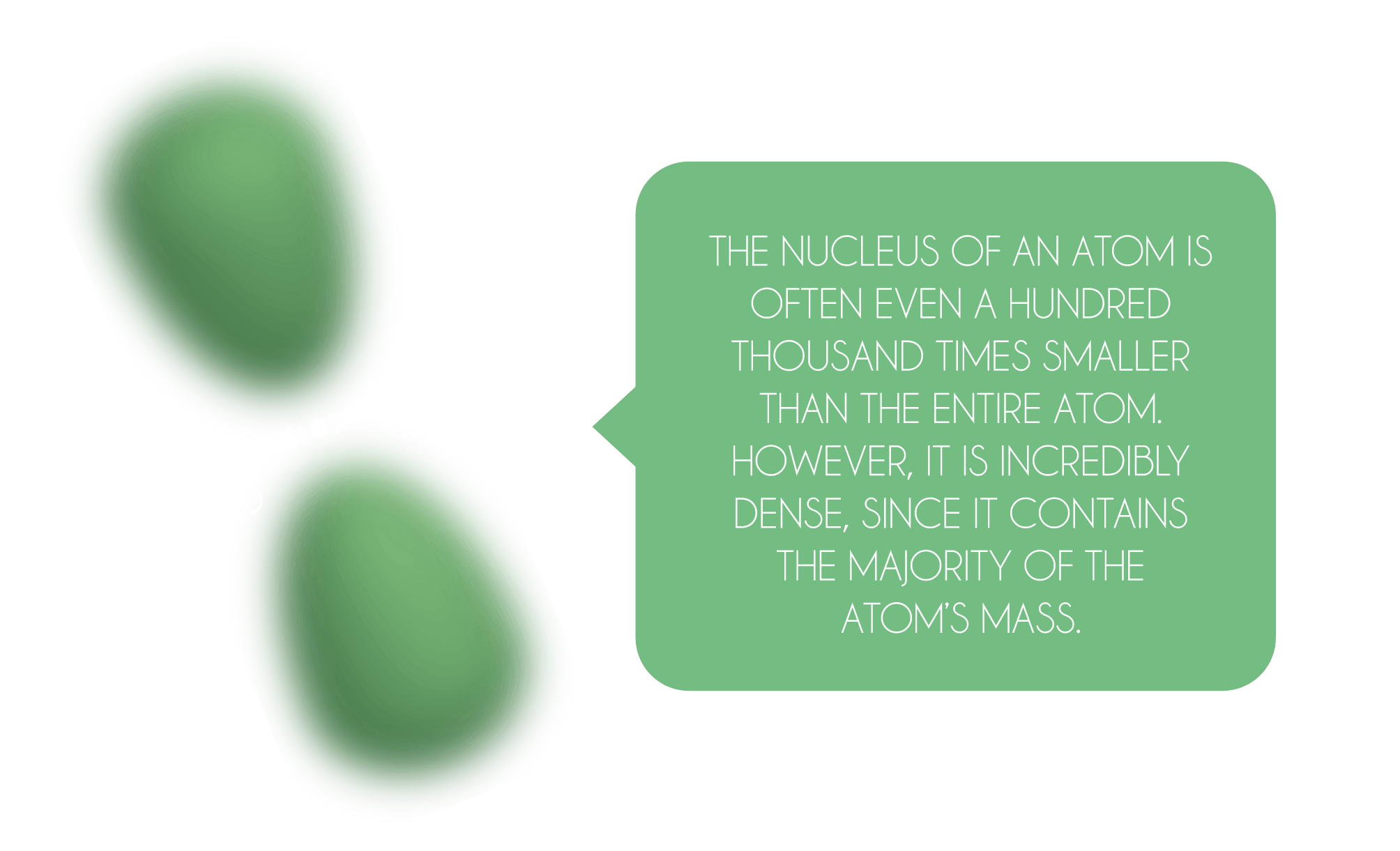
If we managed to squeeze out all of this empty space from atomic shells, we would get matter so concentrated that the entire human race would fit into the volume of a sugar cube. Luckily, I have good news for those who value their personal space and would not appreciate being squeezed into the volume of one cubic centimetre with seven billion fellow citizens – to squeeze out the empty space from atoms seems to be almost impossible. Electrons in the shells are very solitary particles that they would do anything to keep their distance from other electrons. 8 Therefore, if you wanted to convince electrons to move into the nucleus, you would require truly extreme conditions – and from here, we can come back to our supernova, which is the only known object in the universe that is able to provide such conditions.
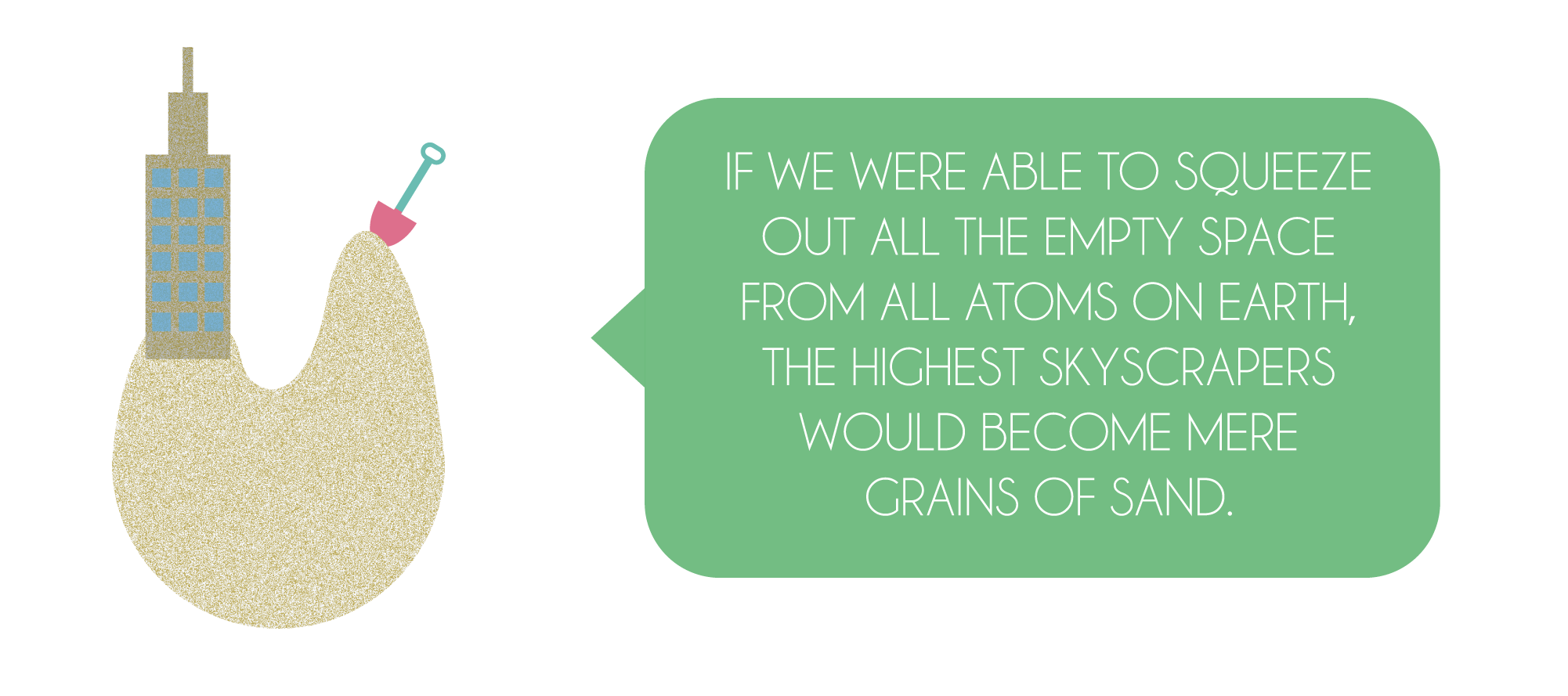
The only thing left after a supernova explosion is a tiny astral core. However, there is a huge gravity in such a core – so huge, in fact, that it starts competing with the immense repulsiveness of electrons. Eventually they resign and are squeezed into the core, where they are along with protons turned into neutrons. The entire stellar core therefore turns into a gigantic sphere of neutrons crammed next to each other, held together by the impressive power of gravity. This formation has earned an apt nickname – a neutron star.
Neutron stars are indeed extreme objects. Their density is so huge that a mere teaspoon of their matter would weight an incredible billion tons. Their radius rarely exceeds 25 kilometres, but they might sometimes be even three times more massive than the Sun (and about a million times more massive than the Earth). On top of that, neutron stars spin very rapidly around their axis, often even 500 times every second – a rotation so unimaginably quick that the human eye would by far not be able to perceive it.
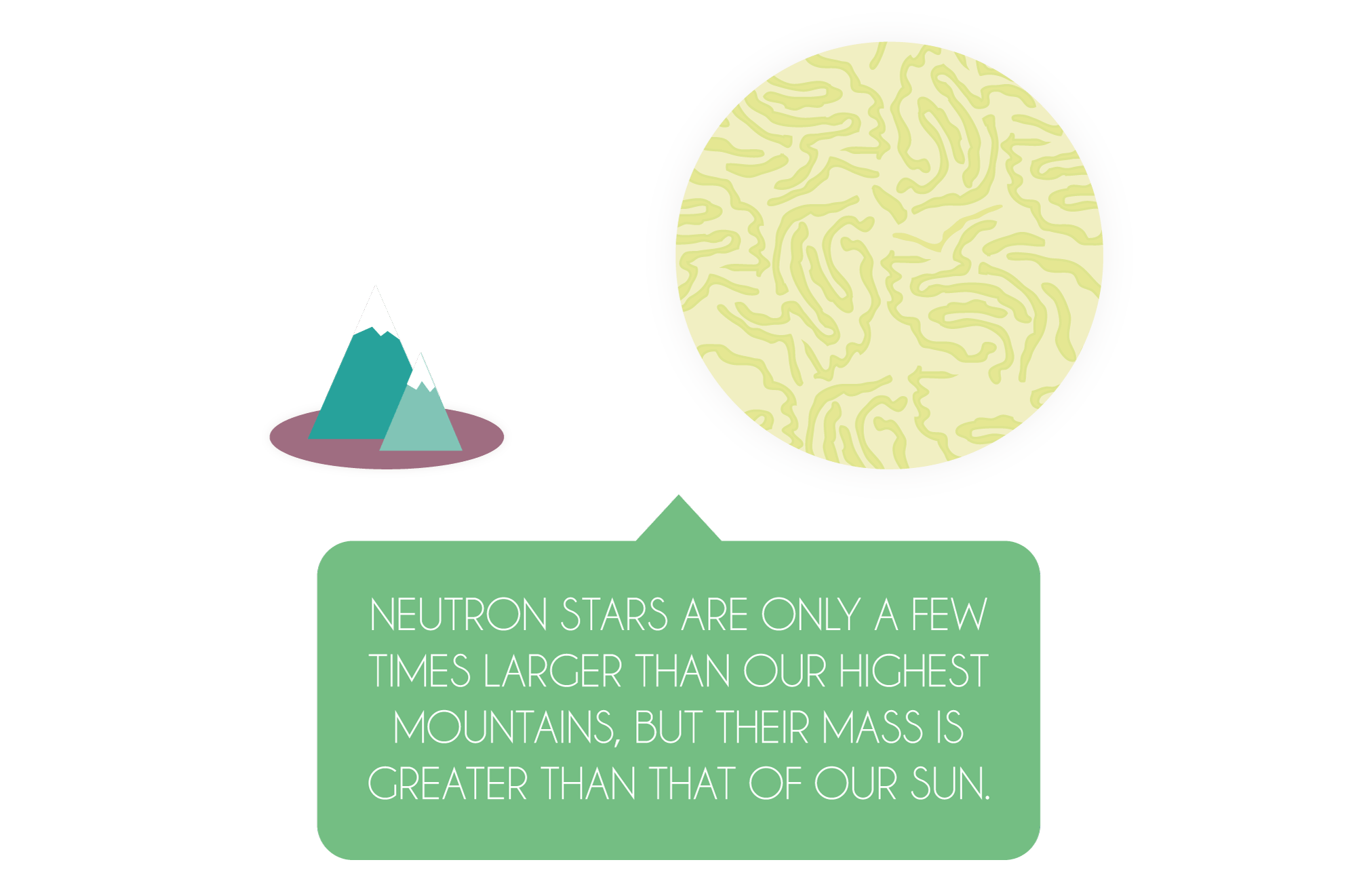
It would be reasonable to assume that neutron stars are definitely the most extreme objects of the cosmos – their rapid rotation and incredible density surely support this claim. However, the laws of the universe allow the existence of an even more extreme type of objects. The existence of such objects was predicted by Einstein’s theory of relativity, but even Einstein himself could not believe that they could be found anywhere in the universe. These objects are called black holes.
If a neutron star created after a supernova explosion is more massive than three Suns, gravity shows its final domination in the universe. It manages to overcome the immense repulsive force of individual neutrons and squeezes the entire neutron star into an infinitely small space called a singularity. We already know this term from the first chapter – the universe used to be squashed into one such singularity before the beginning of time. But the initial singularity was different – nobody had to deal with its gravitational effect on other objects, since it was located in the middle of nothingness. However, this does not apply to singularities formed during a neutron star collapse, whose gravitational influence on the surrounding space-time is simply gigantic. If we were to use the analogy with a trampoline from the chapter about gravity, where every object placed on the trampoline curves its surface, the singularity could be imagined as an object that simply punctures a hole in the trampoline.
This is not good news for any object coming too close to a singularity. Even light itself, the fastest object in the universe, which is rarely influenced by gravity in a significant way, cannot escape a singularity – any light that whizzes around a singularity is ruthlessly pulled inside. That is also the reason why black holes are formed around singularities. A black hole is simply a region of space where the gravitational pull of a singularity is so strong that even light cannot escape it. That logically gives black holes their black colour – it is just impossible to observe an object that does not reflect any light, since it absorbs all of it. The boundary of a black hole is known as the event horizon. What does space inside of a black hole behind the event horizon look like? Nobody knows. Since no light can escape a black hole, no information revealing its appearance can escape either.
There is however one thing we know for certain – falling into a black hole definitely would not count as a pleasant experience. Imagine you are wearing a spacesuit while floating in the proximity of an event horizon and falling towards a black hole at tremendous velocity. As you draw closer and closer, strange things begin to happen. Once you get precisely to the level of the event horizon, you will get a unique opportunity to see your own body from behind. The light reflected off your body can swiftly circle around the entire black hole and consequently get right into your eyes.
But the fall will not be such fun much longer. The crossing of the event horizon will mean your certain death – no matter how much energy you exert, you will never escape the black hole.
Your distance from the singularity will continue to shrink rapidly. Once you get too close, gravity starts doing weird things to your body. If you fall towards the singularity feet first, gravity acting on the lower part of your body is greater than that acting on your upper part. After all, we can see this effect here on Earth as well. If you are standing right now, your body experiences slightly greater gravity than your head. However, this difference is so incredibly small that we do not even feel it.
But that changes inside of a black hole. The difference in gravity is so immense that your body starts happily stretching towards the singularity. The part of your body closer to the singularity therefore starts acquiring unprecedented proportions. This phenomenon has earned an apt name – spaghettification. Eventually, your body is no longer able to counteract the gravitational pressure, since it is obviously not evolutionary built to sustain a fall into a black hole, and tears apart. Your corpus is first split into two parts, then three, four – before you even know it, you are ripped into individual molecules, atoms and consequently even elementary particles, which are then pulled into the singularity. What happens to them then? Unfortunately, nobody knows.
But before being split into trillions of tiny pieces, you will witness some remarkable events. General relativity says that gravity slows down time. Simply said, if you observe somebody in a stronger gravitational field than yourself, you will be able to see them in slow motion. What is more, they will perceive their time completely as normal – form their perspective, their time does not run more slowly, but your time more quickly. This phenomenon takes place here on Earth as well, which also generates a considerable gravitational field. Time would therefore run fractionally more quickly for a person in interstellar space, far away from other large space objects.
But in the immediate vicinity of a black hole, where an enormous gravitational field is present, this effect is definitely not negligible. Your time would slow down in an incredible way near a black hole. Every single of your seconds would be equal to many millions of years here on Earth. The entire age of the universe would therefore literally zoom past your eyes. But after this fantastic experience, the aforementioned dismemberment would follow.
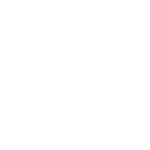
![]() |
![]() |
![]() |
PREVIOUS |
SHARE |
NEXT |
CONCLUSION
And that is all you need to know about stars. Without these omnipresent objects, the universe in its current form would never even exist. Everything you see around you has once been assembled by one of the stars billions of years ago. Every single complex atom of your body used to be in the heart of a star, which was building it for many years by joining lighter elements together. And what is more, all of your atoms, even the atoms of all the other objects on our planet have once been a part of a single star. This massive ancient star eventually exploded as a supernova and ejected its atmosphere into the surrounding cosmos.
The former stellar atmosphere then took the form of a nebula and started collapsing under its own gravitational pull. The Sun was created. Shortly afterwards, the remaining material formed all eight planets of our Solar System, which keep revolving around our parental star to this very day. Almost one billion years thereafter, the first primitive organisms were born. Then, they started evolving diligently for about 3.8 billion years to create me, you and billions of other living organisms that inhabit our beautiful little planet. And all of that was achieved just by using material from a huge ancient star. We could therefore without hesitation proclaim that we come from stars. Literally.
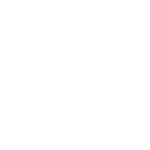
![]() |
![]() |
![]() |
PREVIOUS |
SHARE |
NEXT |